Research
The incredible diversity and heterogeneity of interneurons was observed over a century ago, with Ramon y Cajal hypothesizing in Recollections of My Life that "The functional superiority of the human brain is intimately linked up with the prodigious abundance and unaccustomed wealth of the so-called neurons with short axons." Although interneurons constitute the minority (20%) of neurons in the brain, they are the primary source of inhibition and are critical components in the modulation and refinement of the flow of information throughout the nervous system. Abnormal development and function of interneurons has been linked to the pathobiology of numerous brain diseases, such as epilepsy, schizophrenia and autism. Interneurons are an extremely heterogeneous cell population, with distinct morphologies, connectivities, neurochemical markers and electrophysiological properties. With the advent of new technologies such as single-cell sequencing to dissect gene expression and connectivity patterns, the classification of interneurons into specific subtypes is continuously evolving.
GABAergic interneurons are born in the ventral forebrain during embryogenesis and undergo a prolonged migratory period to populate nearly every brain region. However, our understanding of the developmental mechanisms that generate this diversity of GABAergic cell types remains poorly characterized. The goal of my lab is to dissect the genetic and molecular programs that underlie initial fate decisions during embryogenesis and to explore how the environment and genetic cascades interact to give rise to such a stunning diversity of GABAergic cell subtypes. We take a multifaceted approach, utilizing both in vitro and in vivo strategies to identify candidate mechanisms that regulate interneuron fate decisions. We strive to develop cutting-edge techniques that will overcome the many challenges faced when studying interneuron development. We believe that our pursuits will act as a springboard for future research and provide new insights into both normal development and various neurodevelopmental diseases.
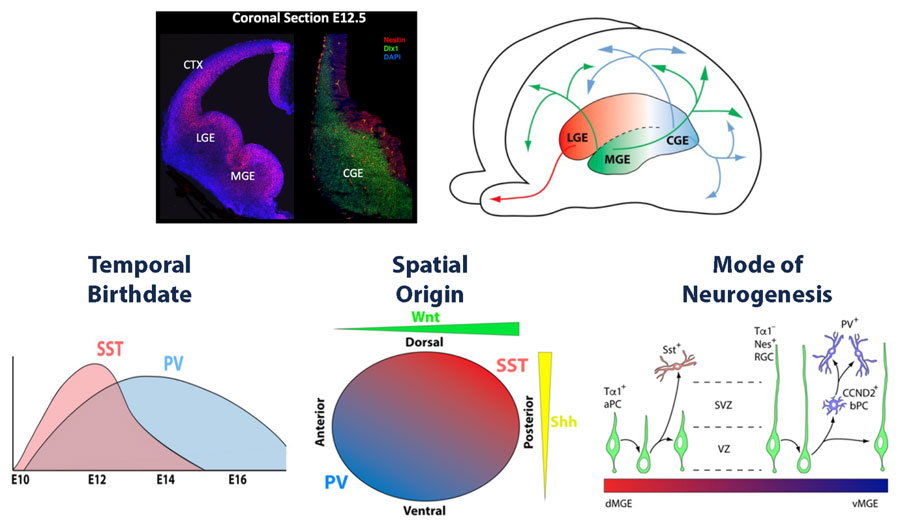
Transcriptional heterogeneity of cycling VZ and SVZ cells throughout the embryonic mouse forebrain
The medial ganglionic eminence (MGE) gives rise to the majority of forebrain interneurons, most notably the somatostatin- and parvalbumin-expressing (SST+ and PV+) subtypes, and nNOS-expressing neurogliaform and ivy cells in the hippocampus. The MGE is a transient, dynamic structure, which arises around E10 and bulges into the lateral ventricle over the next several days before dissipating towards the end of embryogenesis. Given that initial fate decisions are generated within the MGE, there has been much focus on identifying a logic for interneuron generation from this region. Previous experiments characterized both a spatial and temporal gradient within the MGE, which regulates the initial fate decision to become either PV+ or SST+ interneurons. SST+ interneurons are preferentially born early in embryogenesis from the dorsoposterior MGE, whereas PV+ interneurons are born throughout embryogenesis with a bias of originating from the ventroanterior MGE. Previously I discovered an additional mechanism regulating this fate decision: the mode of neurogenesis. Using in utero electroporations, I found that PV+ interneurons are preferentially born from basal progenitors (also known as intermediate progenitors), whereas SST+ interneurons arise more commonly from apical progenitors (Petros…Anderson, Cell Reports ).
Recently, we expanded upon this observation to characterize heterogeneous gene expression in neural progenitors throughout the embryonic forebrain. We performed a comprehensive single-cell RNA sequencing (scRNA-Seq) analysis of ventricular zone (VZ) and subventricular zone (SVZ) cells in four brain regions that give rise distinct cell types: the MGE, LGE (lateral ganglionic eminence), CGE (caudal ganglionic eminence) and cortex. This allows us to compare gene expression in neural progenitors both between distinct brain regions (MGE vs. CGE) and within specific subdomains of these regions (dorsal vs. ventral LGE). We verified many of these gene expression profiles via in situ hybridization and revealed previously unknown transcriptional heterogeneity in VZ cells throughout the forebrain. The findings were recently published (Lee…Petros, eLife ). We are currently following up on several intriguing candidate genes to better understand their role in cell fate.
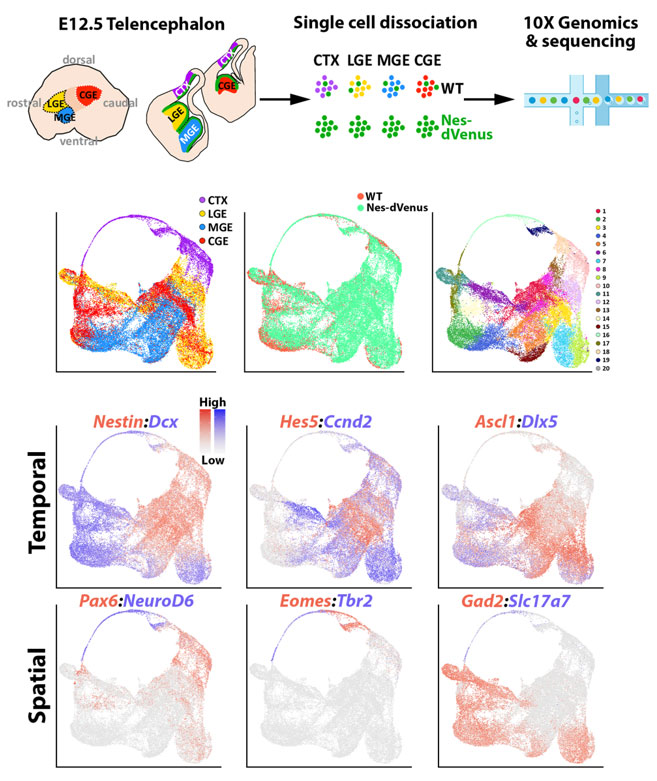
Characterization of the epigenetic landscape during embryonic neurogenesis
In multicellular organisms, cells are genetically homogenous but structurally and functionally heterogeneous as a result of differential gene expression, which is often mitotically heritable. The mechanisms regulating such expression are 'epigenetic,' as they do not involve altering the DNA sequence itself; they include DNA methylation (DNAme), histone modifications, and higher-order chromatin structure. In particular, DNA and histone modifications often follow specific rules termed the 'epigenetic code,' similar to the genetic code. Collectively, DNAme and histone modification have been reported to regulate transcription and chromatin structure in many stem-cell and developmentally critical processes. Previous scRNA-Seq experiments on the ganglionic eminences (GEs) identified surprisingly few region-specific genes in cycling progenitors (immature cells that are still cycling and have not exited the cell cycle), despite the fact that these regions produce distinct GABAergic cell populations. Because there are dynamic changes in the chromatin landscape during development, a prevailing hypothesis is that epigenomic signatures may be a better predictor of cell fate during development, revealing both potential distal enhancers and/or genetic loci that may be 'poised' but not yet expressed. However, direct support for this hypothesis is lacking. The idea is particularly relevant, given that epigenetic changes are observed in many neurological and psychiatric diseases and that most single-nucleotide variants (SNVs) identified in diseases-specific genome-wide association studies (GWAS) map to non-coding regions, implying that epigenetic regulation of gene expression may underlie some disease etiologies.
We performed single-cell assay for transposase-accessible chromatin with sequencing (scATAC-Seq), in combination with Cut&Tag (to characterize histone modifications) and Hi-C/Capture-C (to analyze higher order chromatin interactions), in order to generate an 'Epigenome Atlas' of four different regions in the embryonic mouse brain. This comprehensive, unbiased analysis reveals striking differences in gene expression and chromatin organization between adjacent brain regions and revealed new candidates for region-specific promoter-enhancer interactions that may be critical for neurons' fate and maturation. We are currently following up on several intriguing avenues based on these observations. The work was published earlier this year (Rhodes…Petros, Nat Comm ), and the entire dataset is publicly available in a searchable format on the UCSC Genome Browser platform (https://www.nichd.nih.gov/research/atNICHD/Investigators/petros/epigenome-atlas). Additionally, we recently published a manuscript detailing our procedures to obtain single-cell and single-nuclei dissociations from embryonic and adult mouse brains for numerous downstream applications (Lee…Petros, STAR Protocols
). We are currently examining several mouse models with perturbations in epigenetic processes to better understand how alterations in gene regulation affect interneuron fate and maturation.
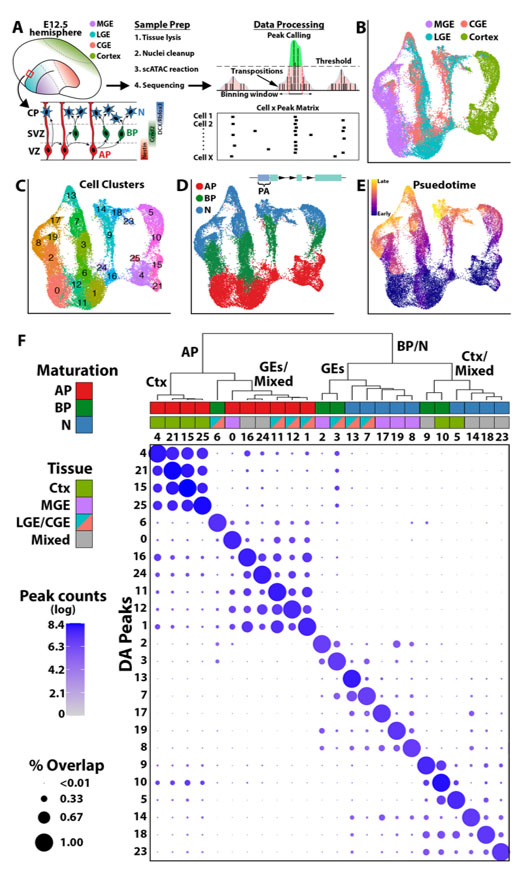
Mechanisms regulating fate determination of CGE–derived interneurons
While significant progress has been made characterizing mechanisms regulating initial fate decisions of MGE-derived interneurons, our understanding of CGE-derived interneurons lags significantly. This is in part because we lack genetic tools to specifically target and manipulate CGE-derived cells. There is an expansion of CGE-derived interneuron subtypes in humans and primates compared with mice, so a better understanding of the developmental trajectory of these cells is warranted.
To this end, we are currently performing several experiments to better understand the developmental logic of CGE-derived cells. We previously performed cell transplant assays of postmitotic MGE-derived interneuron precursors to better understand how the brain environment influences cell fate (Quattrocolo…Petros, Cell Reports ; Quattrocolo…Petros, JoVE
). We are currently performing similar cell transplantation experiments to determine whether specific CGE-derived interneuron subtypes are derived from distinct regions of the CGE. By combining this spatial logic with the scRNA-Seq and scATAC studies detailed above, we hope to link early transcription and chromatin accessibility profiles in CGE progenitors with mature interneuron fates. Second, we are developing a Perturb-Seq approach using mESCs to identify activation (or repression) of genes that promote a CGE fate. Third, based on our insights from the scRNA-seq experiments above, we are generating new transgenic mouse models with the goal of specifically targeting CGE-derived interneurons. In combination, this series of experiments should significantly increase our understanding of mechanisms regulating CGE-derived interneurons.
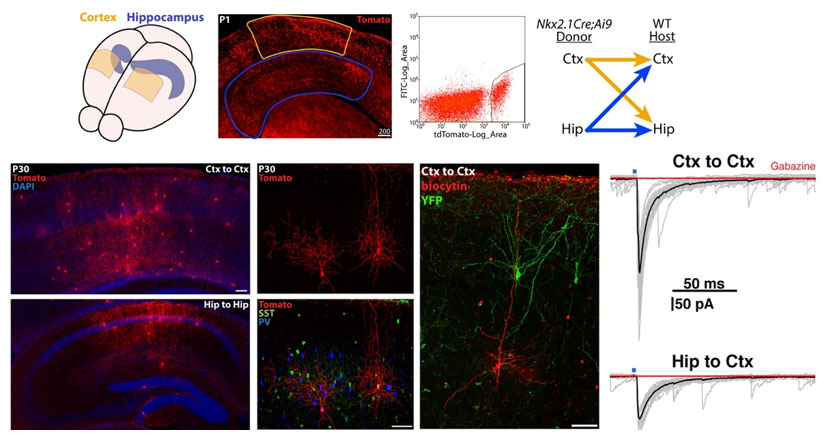